Abstract
Muscle excitability is an experimental technique that probes the properties of the muscle fibre membrane in vivo. In doing so, one can make determinations about the excitability of the membrane during different phases of the muscle velocity recovery cycle, which in turn allows for the assessment of membrane ion channel function. This has been applied to a range of nerve and muscle conditions. To date, it has primarily been used to provide a better understanding of the underlying disease mechanisms and therefore is of relevance to physicians with an interest in neuromuscular conditions. Due to the high intra-individual repeatability and sensitivity of the test, interest is growing in its potential uses as a disease biomarker in therapeutic trials for patients with nerve and muscle diseases.
The assessment of muscle excitability is not an entirely new concept. Standard needle electromyography techniques do measure the presence of spontaneous activity such as fibrillations or positive sharp waves, which provide a very rudimentary assessment of muscle hyperexcitability. This assessment is superficial and does not provide insight into the underlying cellular or electrochemical mechanisms underlying these changes. More useful mechanisms of muscle excitability assessment are now employed in the research setting, and therefore it is useful for physicians interested in neuromuscular disorders to have a basic understanding of these techniques and their utility.
Methods of assessing nerve excitability have been well established on the back of an explosion of research interest in the late 1990s and early 2000s. A detailed description of these techniques is beyond the scope of this article and can be found in Ng & Burke [1] in this journal as well as recent consensus guidelines by Kiernan et al [2]. These techniques can’t be readily applied to muscle for a variety of reasons. Z’Graggen and Bostock developed a recording protocol inspired by the microneurography studies of C-fibres using velocity recovery cycles to facilitate more sophisticated assessments of muscle fibre excitability [3,4]. These techniques have been further refined and can now be performed using a standardised protocol run by the same software (QtracS) often used for nerve excitability assessments.
To perform muscle excitability recordings, a stimulating needle electrode (cathode) is inserted into the muscle of interest. This should be placed distal to the muscle endplate region to avoid unintentional motor axon stimulation. A surface electrode (anode) is placed distally. A small current is applied directly through these electrodes to generate muscle fibre action potentials (MFAPs) in a small number of fibres in the vicinity of the needle electrode. A further recording needle electrode is inserted 2 cm proximally to record from the same group of muscle fibres (Figure 1). It is very difficult to stimulate and record from the same single muscle fibre, but recording a compound muscle fibre action potential from a small number of adjacent muscle fibres is more achievable. The procedure is well tolerated. Apart from the minor discomfort associated with initial needle insertion, most subjects are unable to detect the applied electrical stimuli.
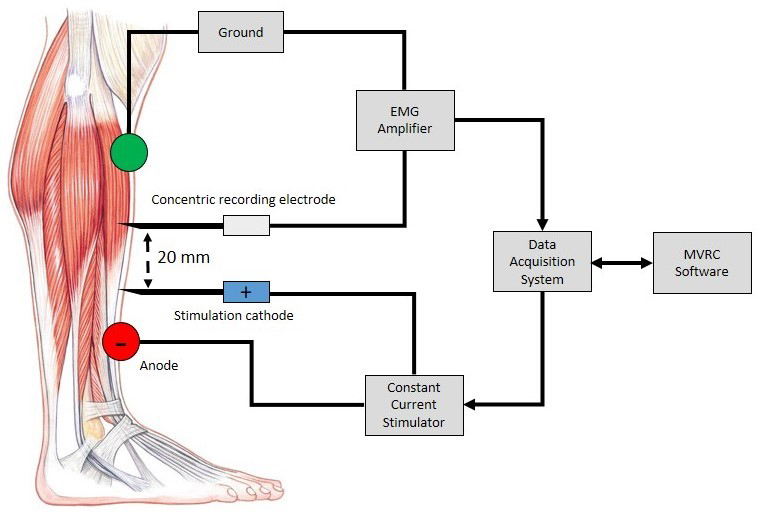
In nerve excitability studies, the primary variable measured is the stimulus amplitude required to generate an action potential that reaches a defined threshold. In muscle excitability recordings, the major component of the assessment is the acquisition of the muscle velocity recovery cycle (MVRC) [3]. The primary variable measured is the change in the MFAP latency. The MFAP latency reflects the conduction velocity of the muscle fibre membrane, which relates to muscle excitability. The standard practice is to measure changes in MFAP latency in response to 1 to 5 preceding conditioning stimuli (10 ms apart) with a varying interstimulus interval.
In normal subjects, we observe that the MFAP latency progressively shortens with shorter interstimulus intervals, reflecting increased MFAP velocity (supernormality) (Figure 2). Supernormality progressively increases until the muscle relative refractory period (MRRP) is reached. Early supernormality (ESN) is thought to be due to the depolarising afterpotential which follows an action potential and reflects the dissipation of charge across the sarcolemma over time [3]. Late supernormality (LSN) is thought to reflect the progressive accumulation of potassium in the sarcolemmal T-tubule system [3].
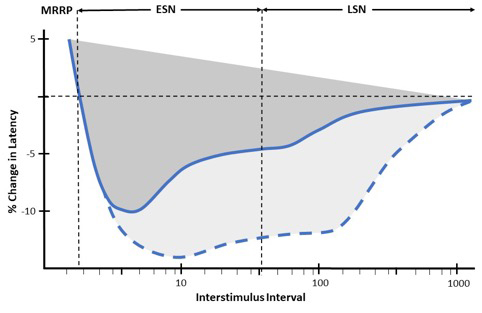
Other common components of the muscle excitability assessment include the frequency ramp and repetitive stimulation protocols. The frequency ramp protocol again measures changes in MFAP latency but does so in response to trains of progressively increasing frequency conditioning stimuli up to 30 Hz [5,6]. The MFAP latency obtained from the final stimulus in the train is compared to the MFAP latency from the initial stimulus to provide a further assessment of sarcolemmal supernormality, which again is thought to be secondary to potassium accumulation within the T-tubule system [5,6]. The repetitive stimulation protocol involves prolonged stimulation at 20 Hz to mimic the short and long exercise tests [5,6]. This is not always performed but can be useful in the assessment of channelopathies.
It is important to note that muscle excitability is affected by several non-pathological variables such as temperature, electrolytes, muscle fibre subtype and patient age. Reduced muscle temperature, particularly when below 30°C, results in an increased MRRP and to a lesser extent reduced supernormality [7]. Electrolyte concentrations are well known to affect membrane dynamics; muscle excitability is particularly dependent on potassium concentrations, with increasing serum potassium, even within the normal physiological range, resulting in an increased MRRP [8,9]. Muscle fibre parameters also vary between different target muscles. This is thought to be secondary to the differential expression of more oxidative type I and IIA fibres in postural muscles and glycolytic type IIX fibres in non-postural muscles. This was demonstrated by Lee et al. who compared the rectus femoris to the tibialis anterior [5,10]. Elderly subjects undergo type II fibre atrophy, which is a possible explanation for the difference in muscle excitability parameters between younger and older subjects [11,12]. Finally, much like explorations of nerve excitability studies, experimental paradigms such as transient ischaemia have been applied to further the understanding of the mechanisms underlying the physiological changes in sarcolemmal depolarisation. When similar changes are seen in pathological conditions, this allows for useful inferences to be made about the underlying mechanisms of disease states [3].
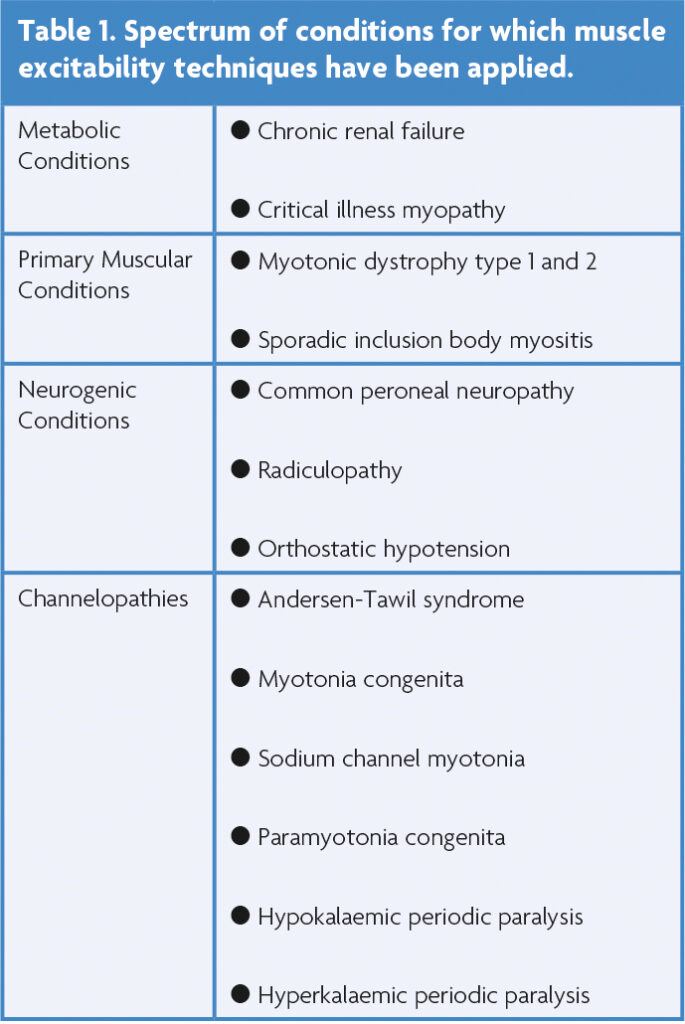
Muscle excitability techniques have been applied to a growing battery of metabolic, myogenic and neurogenic conditions (Table 1). The most commonly assessed muscle is the tibialis anterior due to ease of access, predictable muscle fibre orientation, well-defined motor point and tolerability. The speed and ease of the assessment allowed with the use of the semi-automated QtracS software is a major advantage of the technique. After isolation of a MFAP, the assessment takes less than 20 minutes. However, there are several factors limiting the transition of this technique from the laboratory and into the neurophysiology clinic. Firstly, the assessment requires specialised software, which is not currently available on commonly available electrodiagnostic systems. Furthermore, despite there being good agreement in the measurements from the same type of muscle collected in different laboratories around the world, there is significant inter-individual variability in muscle excitability recordings, and as such, robust normal values do not exist [11]. In contrast, the intra-individual variability is low, making it a sensitive technique for the longitudinal assessment of muscle membrane properties in the same subject over time. Thus, muscle excitability has the potential to become more useful in measuring the response to therapeutic interventions and therefore be a clinical trial biomarker in muscle-based diseases [13].
References
- Ng K, Burke D. Nerve excitability studies in the present era. ACNR 2007; 7(5):29-30.
- Kiernan MC, Bostock H, Park SB, Kaji R, Krarup C, Krishnan AV, Kuwabara S, Lin CS, Misawa S, Moldovan M, Sung J, Vucic S, Wainger BJ, Waxman S, Burke D. Measurement of axonal excitability: Consensus guidelines. Clin Neurophysiol 2020; 131(1):308-323. https://doi.org/10.1016/j.clinph.2019.07.023
- Z’Graggen WJ, Bostock H. Velocity recovery cycles of human muscle action potentials and their sensitivity to ischemia. Muscle Nerve 2009: 39(5):616-626. https://doi.org/10.1002/mus.21192
- Bostock H, Campero M, Serra J, Ochoa J. Velocity recovery cycles of C fibres innervating human skin. J Physiol 2003; 553:649-63. https://doi.org/10.1113/jphysiol.2003.046342
- Boërio D, Z’Graggen WJ, Tan SV, Guetg A, Ackerman K, Bostock H. Muscle velocity recovery cycles: effects of repetitive stimulation on two muscles. Muscle Nerve 2012; 46(1):102-11. https://doi.org/10.1002/mus.23267
- Lee JHF. Muscle and Nerve Excitability in Sporadic Inclusion Body Myositis, in Faculty of Health and Medicine. 2020, University of Sydney.
- Bostock H, Baumann C, Humm AM, Z’Graggen WJ. Temperature dependency of human muscle velocity recovery cycles. Muscle Nerve 2012; 46(2):264-6. https://doi.org/10.1002/mus.23429
- Boland-Freitas R, Lee JHF, Ng K. Serum electrolyte concentrations and skeletal muscle excitability in vivo. Clinical Neurophysiology 2022; 135:13-21. https://doi.org/10.1016/j.clinph.2021.12.008
- Z’Graggen WJ, Aregger F, Farese S, Humm AM, Baumann C, Uehlinger DE, Bostock H. Velocity recovery cycles of human muscle action potentials in chronic renal failure. Clin Neurophysiol 2010; 121(6):874-81. https://doi.org/10.1016/j.clinph.2010.01.024
- Lee JHF, Boland-Freitas R, Ng K. Physiological differences in sarcolemmal excitability between human muscles. Muscle Nerve 2019; 60(4):433-436. https://doi.org/10.1002/mus.26645
- Z’Graggen WJ, Troller R, Ackermann KA, Humm AM, Bostock H. Velocity recovery cycles of human muscle action potentials: repeatability and variability. Clin Neurophysiol 2011; 122(11)2294-9. https://doi.org/10.1016/j.clinph.2011.04.010
- Lee JHF, Boland-Freitas R, Ng K. Sarcolemmal excitability changes in normal human aging. Muscle Nerve 2018; 57(6):981-988. https://doi.org/10.1002/mus.26058
- Ruijs TQ, Koopmans IW, de Kam ML, Tannemaat MR, Groeneveld GJ, Heuberger JAAC. Muscle velocity recovery cycles as pharmacodynamic biomarker: Effects of mexiletine in a randomized double-blind placebo-controlled cross-over study. Clin Transl Sci 2022; 15(12):2971-2981. https://doi.org/10.1111/cts.13418